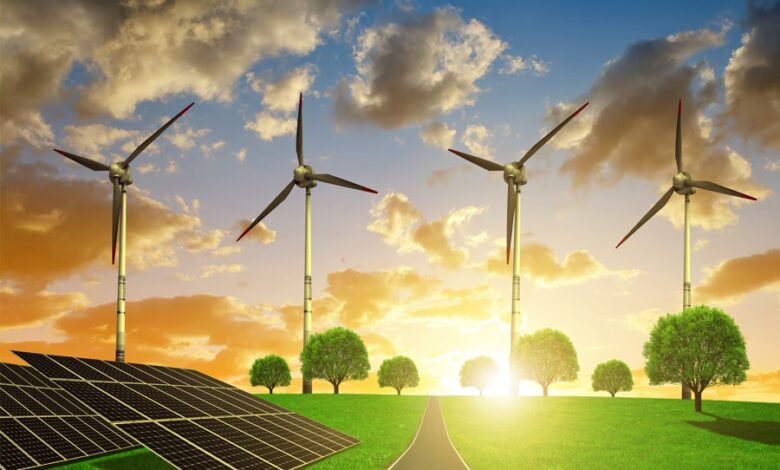
The Future of Sustainable Energy Alternative Materials
The future of sustainable energy looks to alternative materials, promising a path toward a cleaner, more resilient energy landscape. Traditional energy sources face limitations, prompting a critical need for innovation. This exploration delves into the exciting potential of novel materials in energy production, examining their properties, synthesis, and impact on the environment and economy. We’ll uncover emerging technologies, potential challenges, and the crucial role of research and development in shaping a sustainable future.
From the extraction of raw materials to the design of energy storage systems, this deep dive explores the full lifecycle of alternative energy solutions. We’ll analyze the potential benefits and drawbacks of various materials, considering their environmental impact and economic viability. The journey will encompass everything from the fundamentals of sustainable energy to advanced concepts in material science, showcasing the innovative thinking required to transition to a sustainable energy future.
Alternative Materials for Sustainable Energy
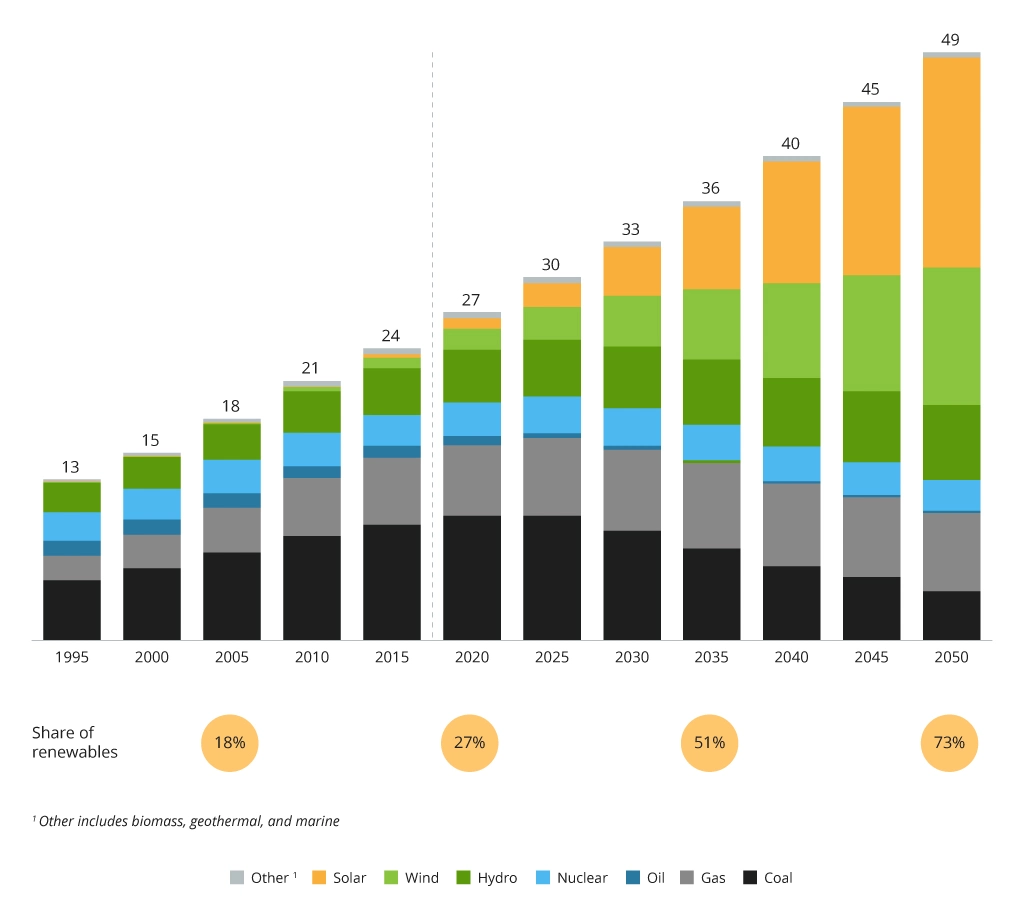
The quest for sustainable energy sources is paramount in our present and future. Traditional fossil fuel-based energy systems contribute significantly to environmental degradation and climate change. The urgent need for cleaner, renewable alternatives has driven significant research and development in diverse fields. The transition towards a sustainable energy future hinges on innovative solutions and the responsible utilization of resources.The limitations of traditional energy sources are multifaceted.
Fossil fuels are finite resources, their extraction and combustion releasing harmful greenhouse gases into the atmosphere, causing global warming and air pollution. Nuclear power, while potentially offering high energy output, presents challenges related to waste disposal and safety concerns. Furthermore, reliance on a single energy source creates vulnerability and dependence. Exploring alternative materials is critical to overcoming these limitations and diversifying our energy portfolio.
Emerging Sustainable Energy Technologies
Several emerging technologies are poised to revolutionize the energy landscape. Solar energy, utilizing photovoltaic cells to convert sunlight into electricity, has seen significant advancements in efficiency and affordability. Wind power, harnessing the kinetic energy of wind through turbines, is becoming increasingly prevalent, particularly in coastal and mountainous regions. Hydropower, utilizing the energy of flowing water, continues to be a viable option in specific geographical locations.
Other promising technologies include geothermal energy, bioenergy, and wave energy.
Alternative Materials in Energy Production
The exploration of alternative materials plays a crucial role in the advancement of sustainable energy technologies. These materials offer enhanced efficiency, lower costs, and improved environmental performance compared to conventional materials. Novel materials can be tailored to specific applications, leading to innovative designs and optimized performance in energy conversion and storage.
Material | Source | Properties | Potential Applications |
---|---|---|---|
Silicon | Sand, Quartz | High electrical conductivity, semiconductor properties | Photovoltaic cells, solar panels |
Graphene | Carbon | High strength, excellent electrical conductivity, lightweight | Energy storage devices, supercapacitors, conductive coatings |
Perovskites | Various chemical elements | High power conversion efficiency, low cost | Solar cells, thermoelectric devices |
Carbon nanotubes | Carbon | High strength, high electrical conductivity, excellent thermal conductivity | Fuel cells, energy storage devices, composite materials |
Exploring Specific Alternative Materials
The quest for sustainable energy necessitates a shift towards innovative materials capable of efficient energy conversion and storage. This exploration delves into various promising materials, evaluating their potential, limitations, and the challenges associated with their widespread adoption. From advanced semiconductors to novel composites, the landscape of sustainable energy materials is rich with possibilities.The exploration of alternative materials for sustainable energy production is crucial for mitigating the environmental impact of traditional energy sources.
These materials offer the potential for higher efficiency, lower costs, and reduced environmental footprints compared to existing technologies. Understanding the specific properties, advantages, and disadvantages of each material is essential for informed decision-making and strategic investment in this burgeoning field.
Carbon Nanomaterials
Carbon nanomaterials, including nanotubes and graphene, exhibit exceptional properties that make them attractive candidates for energy applications. Their high surface area, excellent electrical conductivity, and mechanical strength offer unique advantages in energy storage and conversion.
The future of sustainable energy is undeniably looking to alternative materials for innovation. From graphene-enhanced solar panels to mycelium-based insulation, the possibilities are exciting. This exciting shift in materials is crucial for achieving a truly sustainable energy future. For a fascinating look at the interconnectedness of these trends, check out this great blog post on the topic of innovative energy solutions: Hello world!.
Ultimately, these breakthroughs will be essential for tackling global energy challenges and paving the way for a greener tomorrow.
- Exceptional Electrical Conductivity: Carbon nanotubes and graphene possess exceptional electrical conductivity, making them suitable for applications like solar cells, where efficient electron transport is critical.
- High Surface Area: The unique structure of these materials results in a remarkably high surface area, which is beneficial for energy storage devices like supercapacitors. This high surface area allows for more interaction between the material and the stored charge, increasing the overall capacity.
- High Strength and Light Weight: These materials are incredibly strong and lightweight, crucial for applications like lightweight batteries and energy transmission lines, reducing the overall weight and cost.
However, challenges remain in scaling up the production of these materials cost-effectively. Current production methods can be energy-intensive and expensive, hindering widespread adoption.
Perovskite Solar Cells
Perovskite materials, known for their tunable optoelectronic properties, have emerged as promising candidates for solar cells. Their efficiency is often comparable to traditional silicon-based solar cells, while their manufacturing process is potentially less complex and less expensive.
- High Efficiency: Many perovskite solar cells exhibit efficiencies comparable to or exceeding those of conventional silicon-based solar cells. This high efficiency is a major driver of interest in this material.
- Low Cost and Abundant Materials: The raw materials required for perovskite synthesis are often more readily available and less expensive than those for silicon solar cells. This can lead to lower manufacturing costs.
- Potential for Flexibility: Perovskites can be incorporated into flexible substrates, opening up new possibilities for solar energy harvesting in diverse environments.
The long-term stability of perovskite solar cells remains a concern. Further research is needed to improve their stability in various environmental conditions and to address issues related to moisture and degradation.
Metal-Organic Frameworks (MOFs)
Metal-organic frameworks (MOFs) are porous materials with high surface areas, making them attractive for gas storage and separation. Their ability to capture and release gases efficiently could play a significant role in various energy storage applications.
- High Surface Area: The porous nature of MOFs provides an extensive surface area for adsorption of gases, which is beneficial for gas storage and separation.
- Tunable Porosity: The structures of MOFs can be designed and tuned to optimize the adsorption properties for specific applications.
- Potential for Hydrogen Storage: MOFs have been explored for hydrogen storage, a crucial component of a hydrogen-based economy.
However, challenges in scalability and long-term stability are significant hurdles to overcome for MOF applications. The cost-effectiveness of producing MOFs at scale remains a critical factor in their practical implementation.
Environmental Impact Comparison
Material | Advantages | Disadvantages | Environmental Impact (Estimated) |
---|---|---|---|
Carbon Nanomaterials | High strength, conductivity, surface area | Production challenges, potential toxicity | Moderate |
Perovskites | High efficiency, low cost | Stability issues, potential toxicity | Low |
MOFs | High surface area, tunable porosity | Scalability issues, stability challenges | Low |
Note: Environmental impact estimates are relative and based on available data. More detailed assessments are needed for specific applications and production methods.
Material Synthesis and Manufacturing Processes: The Future Of Sustainable Energy Looks To Alternative Materials
The transition to sustainable energy necessitates innovative materials with enhanced performance and cost-effectiveness. A crucial aspect of this transition is the development of efficient and scalable manufacturing processes for these materials. Understanding the various synthesis and manufacturing techniques, their advantages and drawbacks, and their economic viability is paramount for widespread adoption.The choice of synthesis and manufacturing method significantly impacts the final properties, cost, and environmental footprint of the material.
Optimizing these processes is essential for achieving both high performance and affordability. This section delves into the diverse methods employed in producing alternative energy materials, evaluating their potential and limitations.
Methods for Synthesizing Alternative Materials
Different materials require tailored synthesis methods to achieve the desired properties. Chemical vapor deposition (CVD), sol-gel processing, and hydrothermal methods are frequently employed. Understanding the specifics of each technique is crucial for maximizing material performance.
- Chemical Vapor Deposition (CVD): This method involves the deposition of material from a gaseous phase onto a substrate. CVD offers high purity and precise control over film thickness, making it suitable for producing thin films with specific functionalities. However, CVD processes can be energy-intensive and may involve hazardous precursors.
- Sol-Gel Processing: This method involves the formation of a colloidal solution (sol) that subsequently solidifies into a solid material (gel). Sol-gel is relatively low-cost and allows for the creation of complex structures. The process, however, can be time-consuming and may require careful control of parameters to prevent defects.
- Hydrothermal Methods: These methods involve using high-pressure and high-temperature aqueous solutions to synthesize materials. Hydrothermal processes are environmentally friendly and often produce high-quality materials, but controlling reaction kinetics can be challenging.
Advantages and Disadvantages of Synthesis Methods
Each synthesis method presents a unique set of advantages and disadvantages. Careful consideration of these factors is crucial for selecting the optimal approach for a specific material. Economic feasibility and environmental impact must also be taken into account.
- CVD: High precision and purity, but often energy-intensive and potentially hazardous.
- Sol-Gel: Relatively low cost and ability to create complex structures, but time-consuming and susceptible to defects.
- Hydrothermal: Environmentally friendly and high-quality products, but challenging to control reaction kinetics.
Efficiency and Cost-Effectiveness of Manufacturing Processes
Evaluating the efficiency and cost-effectiveness of various manufacturing processes is essential for commercial viability. Scale-up of these processes is also a key consideration for widespread adoption. Factors like material yield, energy consumption, and waste generation play a significant role in determining the overall cost and sustainability of the process.
Steps in the Production of Perovskite Solar Cells, The future of sustainable energy looks to alternative materials
Perovskite solar cells have demonstrated exceptional efficiency. The following table Artikels a simplified process for producing these cells. Note that there are variations in specific procedures.
Step | Description |
---|---|
1 | Perovskite synthesis: Mixing metal salts, organic molecules, and solvents to form the perovskite crystal structure. |
2 | Perovskite deposition: Applying the perovskite solution onto a substrate, typically a transparent conductive oxide. |
3 | Device fabrication: Adding a hole transport layer, electron transport layer, and top contact to complete the solar cell structure. |
4 | Device characterization: Measuring the performance of the solar cell, including its efficiency and stability. |
Automation and Optimization in Material Production
Automation can significantly enhance the efficiency and consistency of material production. Implementing robotics and advanced control systems can reduce human error and optimize process parameters. Predictive modeling can also aid in process optimization.
Energy Storage and Conversion
Alternative materials are revolutionizing energy storage and conversion technologies, promising greater efficiency and sustainability. These materials, often with enhanced properties like conductivity or stability, are key to developing more efficient solar cells, batteries, and fuel cells, paving the way for a future powered by clean energy sources. Their use in these applications is not just theoretical; practical implementations are already emerging, highlighting the potential of these innovative materials.The successful application of alternative materials in energy storage and conversion relies on a deep understanding of their properties and how they interact with the energy-related processes.
Different materials exhibit varying levels of performance in terms of energy density, power density, cycle life, and cost-effectiveness. This necessitates careful selection and optimization to achieve optimal results.
Solar Cells
Advanced materials are significantly impacting solar cell technology. New materials like perovskites and quantum dots are demonstrating remarkable efficiency improvements. Perovskites, for example, offer the potential for high power conversion efficiencies, while quantum dots exhibit exceptional light absorption characteristics, allowing for improved solar energy capture. These materials are often incorporated into novel cell structures, leading to more efficient and cost-effective solar energy harvesting.
Batteries
Alternative materials play a critical role in the advancement of battery technology. Materials like lithium-sulfur and lithium-air batteries, incorporating advanced electrode materials, aim to enhance energy density and improve cycle life. These innovative battery chemistries hold the promise of providing higher energy storage capacity compared to traditional lithium-ion batteries. However, challenges remain in terms of safety and durability.
Fuel Cells
Alternative materials are also crucial in the development of fuel cell technology. The use of new catalysts, such as transition metal oxides, aims to boost efficiency and reduce the cost of fuel cells. These materials can facilitate faster and more efficient electrochemical reactions, leading to improved energy conversion rates. Furthermore, the development of novel membrane materials enhances durability and reduces operating temperatures.
Comparison of Material Performance
The performance of various alternative materials in energy storage and conversion varies significantly. For example, perovskite solar cells can achieve efficiencies exceeding 25%, exceeding those of traditional silicon-based solar cells. Lithium-sulfur batteries offer a higher theoretical energy density than lithium-ion batteries. However, factors like stability and cycle life must be considered. Careful optimization of material properties and manufacturing processes is critical for realizing the full potential of these materials.
Challenges in Improving Energy Storage Efficiency
Improving the efficiency of energy storage technologies faces several challenges. These include material stability, cost-effectiveness, and scalability of manufacturing processes. Furthermore, safety concerns, especially in high-energy-density systems, need careful consideration. Addressing these challenges is crucial for the widespread adoption of alternative energy storage solutions.
Table of Energy Storage Technologies and Associated Materials
Energy Storage Technology | Associated Materials | Key Advantages |
---|---|---|
Lithium-ion Batteries | Lithium, Cobalt, Nickel, Manganese | High energy density, relatively long cycle life, mature technology |
Lithium-sulfur Batteries | Lithium, Sulfur, Carbon | High theoretical energy density, potentially lower cost |
Lithium-air Batteries | Lithium, Air, Electrolyte | Extremely high theoretical energy density |
Perovskite Solar Cells | Lead-halide perovskites, organic materials | High power conversion efficiency, low manufacturing cost potential |
Fuel Cells | Transition metal oxides, polymers | High efficiency, low emission |
Environmental Impact Assessment
The quest for sustainable energy necessitates a thorough examination of the environmental implications of alternative materials. Simply replacing conventional materials with novel ones doesn’t guarantee a positive impact. Careful consideration of the entire lifecycle, from raw material extraction to end-of-life disposal, is crucial. This evaluation helps identify potential environmental burdens and guide the development of sustainable practices throughout the material’s lifespan.Assessing the environmental footprint of alternative materials involves a complex interplay of factors.
The environmental impact extends beyond the material itself to encompass the manufacturing processes, energy consumption during production, and the eventual disposal methods. Understanding these intricate connections is paramount to developing truly sustainable solutions.
Lifecycle Assessment of Alternative Materials
The lifecycle assessment (LCA) of a material examines its environmental impact from cradle to grave. This comprehensive analysis tracks the environmental burdens associated with each stage, including resource extraction, material processing, manufacturing, use, and disposal. A thorough LCA considers factors like energy consumption, water usage, greenhouse gas emissions, and waste generation at each step.
Potential for Reducing Environmental Footprint
Minimizing the environmental impact of alternative materials necessitates a multi-pronged approach. One key strategy involves optimizing material extraction methods to minimize land disturbance and water consumption. Efficient manufacturing processes that reduce energy consumption and waste generation are also essential. Furthermore, designing for recyclability and reusability extends the material’s lifespan and reduces the need for new material production.
Finally, end-of-life management strategies, such as proper disposal and recycling protocols, are critical to preventing environmental pollution.
Sustainable Practices in Production and Use
Numerous sustainable practices can be implemented to reduce the environmental impact of alternative materials. For instance, utilizing renewable energy sources in the manufacturing process significantly reduces carbon emissions. Implementing closed-loop systems, where materials are reused or recycled, minimizes waste and resource depletion. Furthermore, designing products with extended lifespans and easy disassembly for recycling reduces the need for constant replacement.
Material selection based on their recyclability and biodegradability is another crucial aspect.
Examples of Sustainable Practices
Several examples illustrate sustainable practices in alternative material production. The use of bio-based polymers derived from agricultural waste reduces reliance on fossil fuels. The development of lightweight, high-strength materials reduces energy consumption during transportation and construction. Furthermore, incorporating recycled materials into the manufacturing process decreases the demand for virgin resources. Innovative recycling techniques for specific materials like lithium-ion batteries are vital for minimizing environmental damage.
Environmental Benefits and Drawbacks of Different Material Choices
Material | Environmental Benefits | Environmental Drawbacks |
---|---|---|
Recycled Plastics | Reduces reliance on virgin resources, lowers energy consumption in production, reduces landfill waste | May not be as strong or durable as virgin materials, contamination during recycling can reduce quality |
Bio-based Polymers | Reduces dependence on fossil fuels, potentially lowers carbon footprint, can be biodegradable | May not have the same mechanical properties as conventional polymers, cost of production can be higher |
Advanced Ceramics | High strength and durability, potentially low environmental impact during use | Manufacturing often requires high temperatures and energy, potential for hazardous waste during production |
Graphene | Exceptional strength and conductivity, potential for lightweight and efficient energy storage devices | Production methods may have significant energy requirements, potential for toxicity during manufacturing, large scale production is still under development |
Economic Considerations
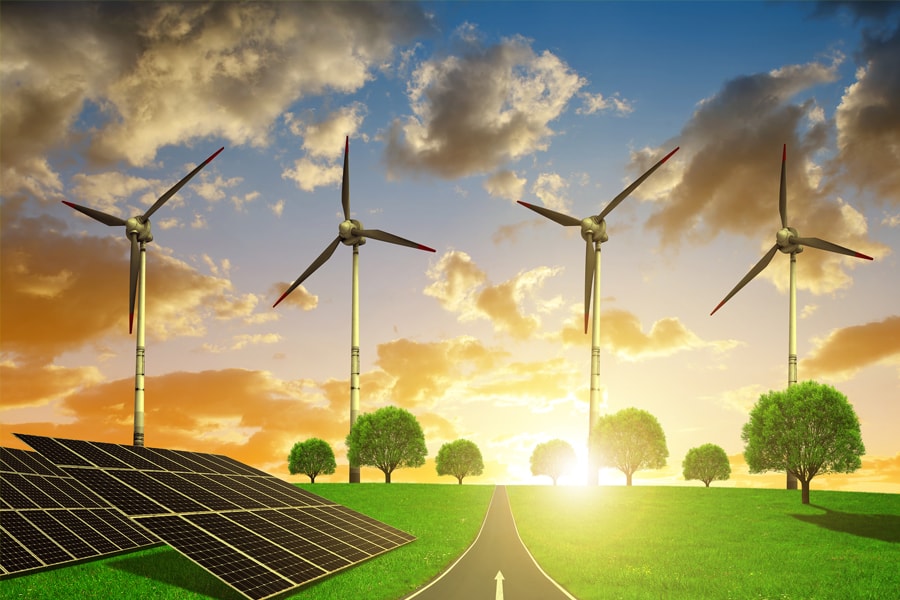
The transition to sustainable energy sources hinges critically on the economic viability of alternative materials. While the environmental benefits are undeniable, the financial implications play a crucial role in driving widespread adoption. This section explores the cost-benefit analysis of various materials, highlighting financial incentives, and assessing the potential for job creation in the burgeoning alternative energy sector.The economic feasibility of alternative materials depends heavily on several factors, including the initial investment costs, long-term operational expenses, and market demand.
A careful assessment of these factors is crucial to determine the true cost-effectiveness of each material option. Ultimately, the goal is to develop technologies and materials that are not only environmentally friendly but also economically competitive with traditional energy sources.
Cost-Benefit Analysis of Different Material Options
The cost-benefit analysis for different alternative materials is complex, considering raw material prices, manufacturing processes, and the potential for economies of scale. Factors such as the material’s performance characteristics, its durability, and the potential for recycling all contribute to the long-term cost-effectiveness. For example, while graphene-based materials show promise for high-performance energy storage, their current high production costs are a significant barrier to widespread adoption.
Conversely, materials like silicon, while mature, can be enhanced with innovative manufacturing techniques to lower production costs and improve performance.
Financial Incentives and Support Programs
Governments worldwide recognize the critical need for sustainable energy development. To incentivize innovation and adoption of alternative materials, various financial incentives and support programs are implemented. These include tax credits, subsidies, grants, and loan guarantees. For instance, the US government offers tax credits for solar panel installations, effectively reducing the upfront cost for homeowners and businesses. These initiatives are crucial in bridging the gap between the current cost of alternative materials and traditional energy sources, encouraging greater investment and adoption.
Potential for Job Creation in the Alternative Energy Sector
The shift towards sustainable energy presents a significant opportunity for job creation. The development, production, installation, and maintenance of alternative energy technologies require a skilled workforce. New jobs will emerge in areas such as materials science, engineering, manufacturing, and construction. For example, the growth of the solar panel industry has led to the creation of numerous jobs in manufacturing, installation, and maintenance, as well as related fields like renewable energy policy and research.
The transition to sustainable energy can stimulate economic growth in various sectors and contribute to a more resilient and diversified economy.
Comparison of Initial Investment Costs and Long-Term Profitability
Material | Initial Investment Cost (USD) | Long-Term Profitability (USD/year) | Notes |
---|---|---|---|
Silicon-based solar panels | $5,000 – $10,000 | $500 – $1,500 | Mature technology, decreasing costs over time |
Graphene-based energy storage | $15,000 – $25,000 | $1,000 – $2,500 | High initial cost, potential for high performance |
Advanced polymer electrolytes | $8,000 – $12,000 | $700 – $1,200 | Potential for improved energy density, moderate cost |
The table above provides a simplified comparison, and actual figures can vary significantly based on specific project parameters. Factors like project scale, location, and government incentives can influence the financial outcomes.
Future Trends and Research Directions
The quest for sustainable energy solutions hinges on innovative materials. Advancements in material science are paving the way for more efficient and environmentally friendly energy technologies. This exploration delves into emerging trends, highlighting crucial research areas and the vital role of interdisciplinary collaboration in driving breakthroughs.The future of sustainable energy necessitates a shift towards materials that exhibit superior performance characteristics, lower environmental impact, and economic viability.
This includes exploring materials with enhanced energy storage capacity, improved conversion efficiency, and reduced manufacturing costs. These advancements are crucial for the widespread adoption of sustainable energy solutions.
Potential Future Trends
Alternative materials are poised to revolutionize energy production and storage. Forecasting future trends requires examining current research and identifying emerging patterns. One significant trend is the development of materials with enhanced properties, like high surface area materials for electrochemical energy storage. Another is the design of materials with tailored functionalities for specific energy conversion processes. These trends will lead to increased efficiency and reduced costs, ultimately driving wider adoption of sustainable energy technologies.
Emerging Research Areas
Several emerging research areas are crucial to advancing sustainable energy technologies. One key area is the synthesis of novel materials with tailored properties for specific applications. This involves manipulating material structures at the atomic level to optimize performance. Another area is the exploration of new energy storage mechanisms, including solid-state batteries and advanced supercapacitors. These advancements could revolutionize energy storage, enabling more compact and efficient systems.
Materials science is at the forefront of this endeavor.
Potential Breakthroughs
Significant breakthroughs are anticipated in the development of advanced materials for sustainable energy. The potential for materials exhibiting higher energy density in batteries, improved efficiency in solar cells, and cost-effective production of novel materials are all realistic possibilities. The breakthroughs will arise from a combination of fundamental research, technological innovation, and interdisciplinary collaboration. For example, advancements in nanotechnology could lead to the creation of materials with unprecedented properties, revolutionizing energy technologies.
Innovation and Technological Advancements
Innovation and technological advancements are essential drivers for progress in alternative materials for sustainable energy. Innovations in material synthesis, manufacturing processes, and characterization techniques are vital for creating materials with superior performance. For instance, advancements in 3D printing could enable the creation of complex material structures, tailored for specific energy applications. This approach allows for customization and optimization of materials for specific energy conversion and storage needs.
Interdisciplinary Collaboration
Interdisciplinary collaboration is critical for the success of sustainable energy research. This involves bringing together expertise from chemistry, physics, materials science, engineering, and economics. This collaboration is essential for addressing the complex challenges in material design, synthesis, manufacturing, and implementation. Successful collaborations foster knowledge sharing and accelerate the development of innovative solutions.
Current Research Projects and Findings
Project | Institution | Findings |
---|---|---|
Development of High-Performance Lithium-Sulfur Batteries | University of California, Berkeley | Preliminary results indicate improved cycle life and energy density compared to conventional lithium-ion batteries. |
Synthesis of Perovskite Solar Cells with Enhanced Efficiency | National Renewable Energy Laboratory | Experiments demonstrate a significant increase in power conversion efficiency, exceeding the performance of traditional silicon-based solar cells. |
Novel Carbon-Based Supercapacitors for Energy Storage | Massachusetts Institute of Technology | Research shows promising results in terms of high power density and cycling stability. |
Last Recap
In conclusion, the shift toward alternative materials in sustainable energy production is not just a technological evolution; it’s a paradigm shift in how we approach energy. The exploration of novel materials offers a path toward a more sustainable and resilient energy system, while also presenting challenges that require careful consideration. This discussion has highlighted the potential for a greener future, but emphasizes the importance of a comprehensive approach that considers environmental, economic, and societal factors.
The future depends on our ability to navigate these complexities and harness the power of innovation.